Projects
Remote Tissue Sensing/Imaging
Current Projects
Tissue Oxygenation Imaging
We aim to design and demonstrate a remote optical tissue oxygenation monitoring system that can map tissue oxygenation values from various distances. We wish to incorporate computational imaging algorithms, optimized hardware (e.g., miniature light sources, light modulators, coherent illumination) to improve system accuracy and motion robustness, reduce bias due to skin color, and extract oxygenation data from variable depths in tissue. This research area is part of a collaboration with the Computational Imaging Group @ UofT, and the Institute of Optics (INO) in Quebec City, QC.
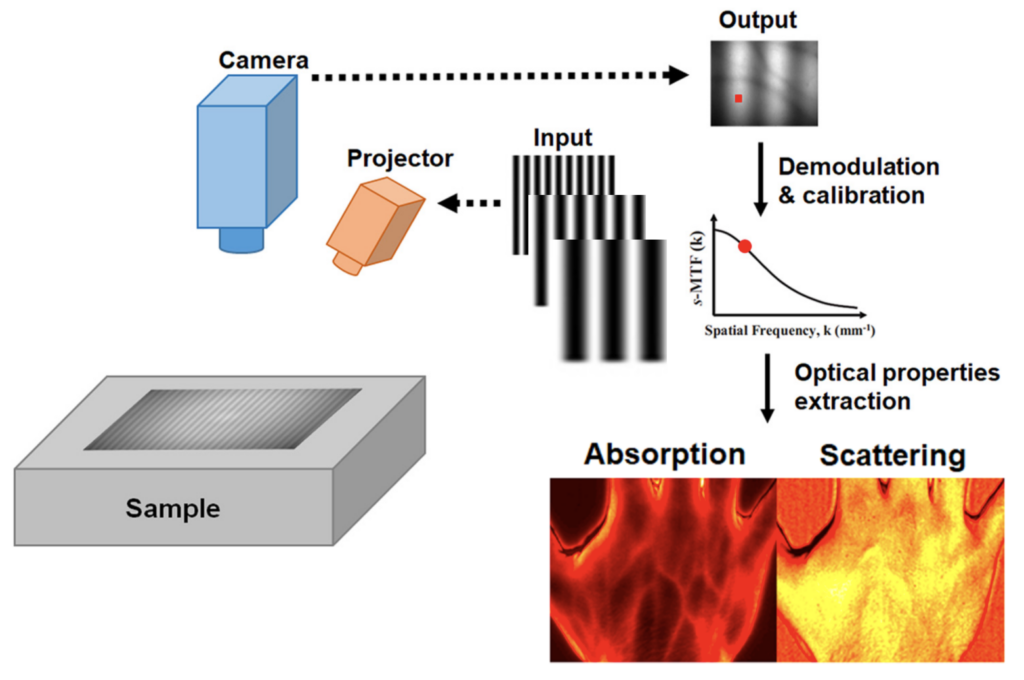
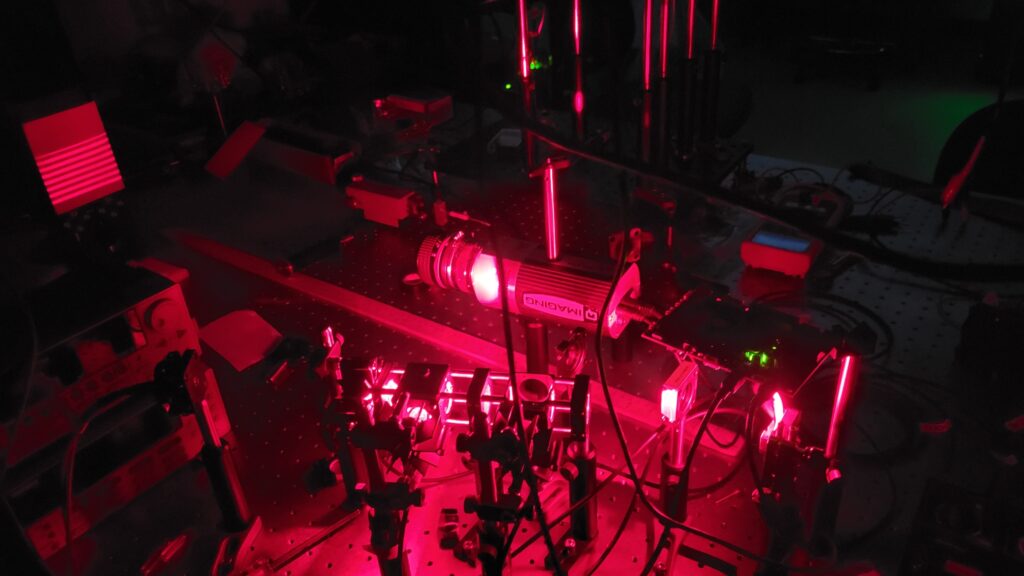
Left: Typical SFDI setup adapted from [1]. Right: SFDI setup in our lab.
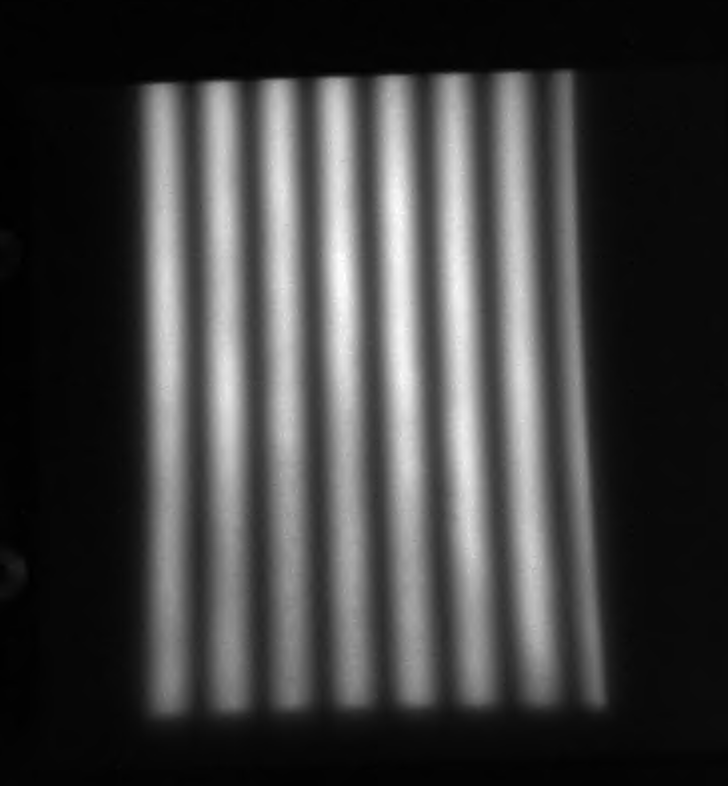
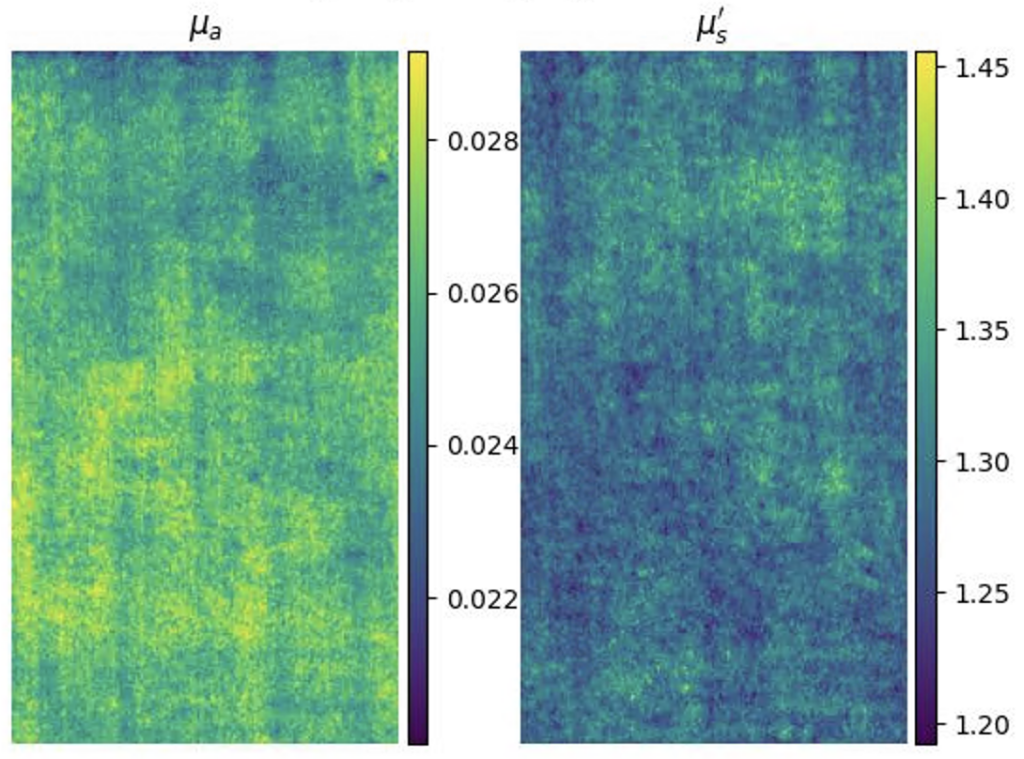
Left: Raw SFDI image of tissue phantom taken in lab. Right: Recovered optical properties.
References
- S. Gioux, A. Mazhar, and D. J. Cuccia, “Spatial frequency domain imaging in 2019: principles, applications, and perspectives,” J Biomed Opt 24, 071613 (2019).
Past Projects
Remote Vital Signs Monitoring
Remote monitoring of vital signs provides a low-cost, non-intrusive method of assessing an individual’s health. These systems have wide-ranging applications, including detection of patient deterioration in clinical settings and home monitoring of at-risk individuals. We developed a novel remote vital signs monitoring system that integrates 3-D depth information and light intensity data to increase tolerance to subject motion. We used 3-D depth analysis and measurement of reflected light intensity due to blood volume variations to estimate heart rate and respiratory rate, two important vital signs. This method achieves a mean error of 1.7 beats per minute for heart rate and 0.05 breaths per minute for respiratory rate. Furthermore, techniques are also described to quantify the severity of motion artifacts, and methods of motion correction are applied to salvage physiological data from noisy signals. Future work will include examining additional data scenarios and improving analysis methods and motion tolerance.
(Text adapted from [1]).
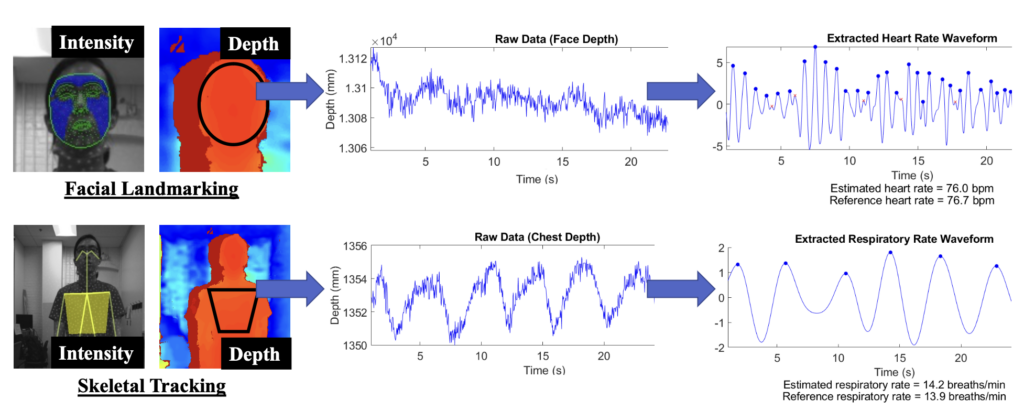
References
- R. Chu. “Remote Vital Signs Monitoring with Depth Cameras.” Thesis. 2020.
- R. Chu, L. Kuramoto, D. Ringuette, E. Y. Zhu, and O. Levi, “Correlation of Near-Infrared Intensity and Depth Channels for Remote Vital Signs Monitoring ”, SPIE Photonics West 2021 conference 11651, Optical Diagnostics and Sensing XXI: Toward Point-of-Care Diagnostics, paper 116510F, San Francisco, CA.
Neural Imaging
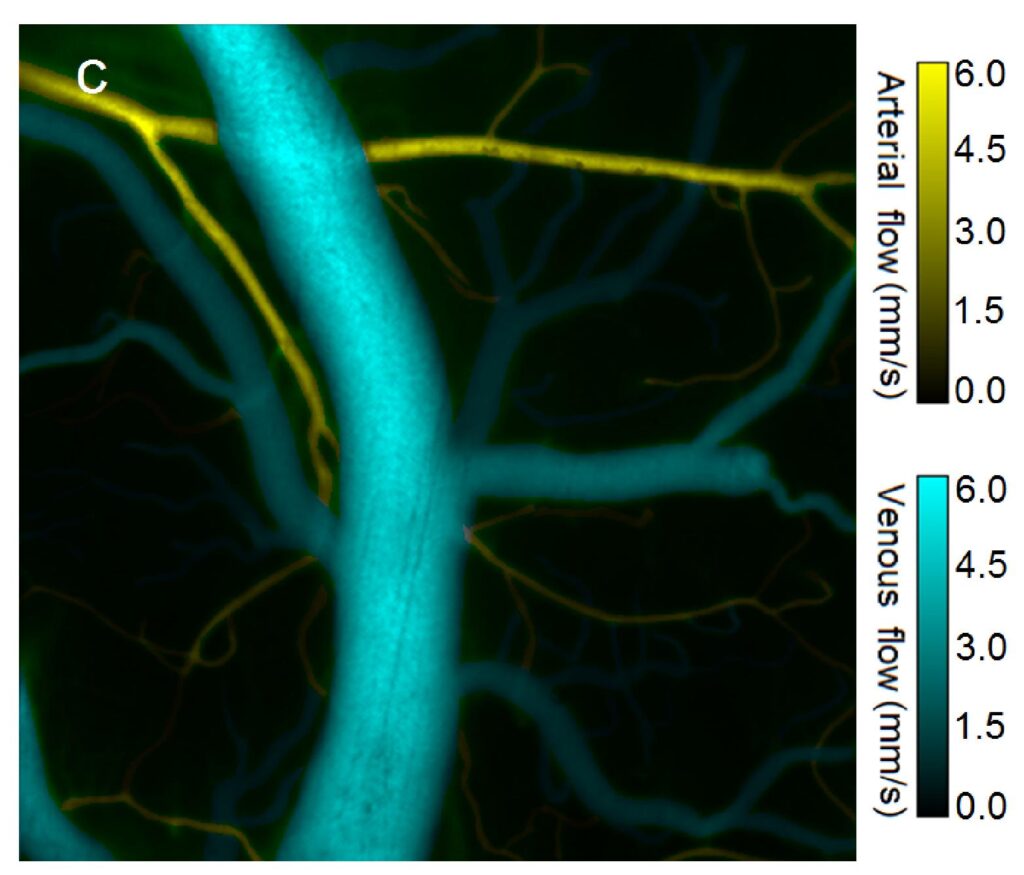
A significant advantage of optical brain imaging over other imaging modalities like fMRI, PET, and CT is that optical systems are low cost, flexibility and small size. These properties allow for several applications, including multispectral tissue imaging and minimally invasive optical coherence tomography imaging. Furthermore, monitoring the activity of neuronal populations is key to understanding the functional architecture of the brain. Optical imaging of hemodynamic correlates to brain activity, known as Intrinsic Optical Signal (IOS) imaging (for blood oxygenation levels) and Laser Speckle Contrast Imaging (LSCI) (for blood flow changes) are widely accepted techniques for obtaining maps of brain function in the cerebral cortex. We have developed multi-modal imaging techniques that use Vertical Cavity Surface Emitting Lasers (VCSELs) as an agile illumination in brain studies. Monitoring the activity of neuronal populations is key to understanding the functional architecture of the brain. Optical imaging of hemodynamic correlates to brain activity, known as Intrinsic Optical Signal (IOS) imaging (for blood oxygenation levels) and Laser Speckle Contrast Imaging (LSCI) (for blood flow changes) are widely accepted techniques for obtaining maps of brain function in the cerebral cortex.
(LSC) Imaging measures relative velocity of blood flow. The scattering of coherent light off tissue produces a random interference pattern known as speckle. The intensity variation of interference fringes is reduced by sample movement as different patterns are superimposed. The speckle contrast ratio (CR) is a measure of fringe intensity which can be related to coherence time, a quantity inversely proportional to velocity. Intrinsic Optical Signal (IOS) Imaging measures reflectance changes from brain tissue resulting from neural activity. The reflection changes are due to changes in blood oxygenation and volume, which are a surrogate to neural activity. Non-coherent light is an ideal light source for tissue reflectance imaging, such as IOS as it avoids noise from scatter-based speckle patterns. A light emitting diode (LED) is a common low noise light source for such measurements. Previous studies have shown that increased noise values due to coherence effects in laser diodes mitigate the benefits of increased brightness they have over LEDs. The spectral linewidth Δλ of a laser can be increased by operating the laser in a “multimode” regime, where multiple transverse modes are supported. The coherence length Lc can be reduced by increasing the spectral linewidth according to:

where λ is the laser wavelength and Δλ is the FWHM spectral width. This change in Lc leads to a reduction in speckle magnitude. Speckle can be further reduced by cycling between different multimode states, blending interference patterns and polarization states while imaging the tissue. Vertical Cavity Surface Emitting Laser (VCSELs) have been shown to be stable and have low values of relative intensity noise (RIN). These low noise lasers were applied for high brightness IOS imaging in addition to LSC imaging. The laser alone can be driven while switching power schemes between frames.
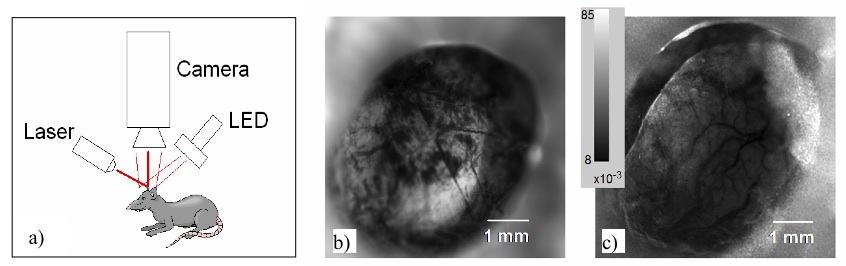
We have demonstrated that changing a VCSEL operation between single mode and current sweep mode can be used to manipulate speckle noise properties of a VCSEL source, allowing us to use a single light source to image a tissue sample via two different modalities. We show that the noise reduction associated with current sweep mode lower the temporal and spatial noise of the VCSEL to values comparable to that of a low noise LED. Comparison of temporal noise values for VCSEL illumination in single mode, in sweep mode, and for the LED operation showed that by applying the current sweep, coherence effects of a VCSEL laser can be reduced to produce noise levels like those of an LED (Fig. NP3). Fig. NP3a shows that temporal contrast can also be used to produce flow topology maps based on speckle variation. In a single mode VCSEL illumination, we observe high noise values and vascular detail even at non- ideal focus plane for capturing blood vasculature. In current sweep VCSEL illumination (Fig. NP3b) the vasculature details are washed away and noise values are reduced significantly compared to single mode illumination. In LED illumination we observe noise values comparable within a factor of 2 to current sweep VCSEL illumination mode, deviation/mean) units and essentially no vascular details are visible. The noise reduction due to a current sweep illumination, corresponds a reduction to the coherence length Lc and increasing the spectral linewidth for the VCSEL light source. Current changes also introduce thermal and polarization changes that support rapid transverse mode sweep. Utilizing this phenomenon, a single laser illumination source under two power schema can support a dual modality imaging system. By taking advantage of the strong coherence effects of the VCSEL in single mode, speckle contrast images can be generated to reveal vasculature. The resulting contrast ratio maps can be converted to speckle correlation time maps, and through use of multiple exposures, this data can be used to directly quantify blood flow. Alternatively, by driving the VCSEL in current sweep mode, we can utilize the VCSEL for imaging as we would use a low noise LED source.
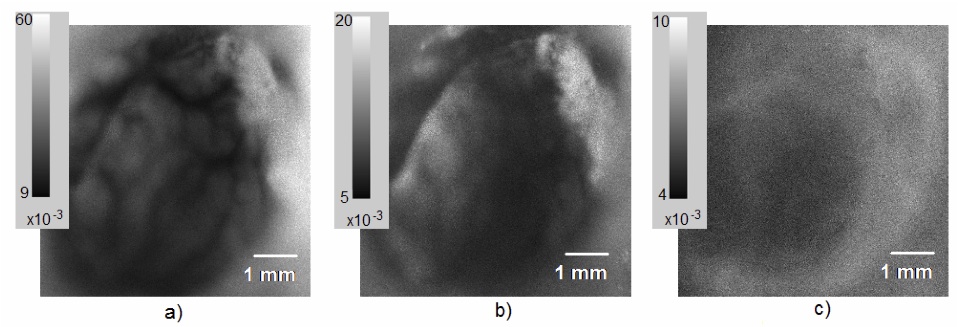
References
- E. A. Munro, H. Levy, D. Ringuette, T. D. O’Sullivan, and O. Levi, “Multi-modality optical neural imaging using coherence control of VCSELs,” Opt. Express 19, pp. 10747-10761 (2011).
- H. Levy, D. Ringuette, and O. Levi, “Rapid monitoring of cerebral ischemia dynamics using laser-based optical imaging of blood oxygenation and flow” Biomed. Opt. Express 3(4), pp. 777-791 (2012).
Advanced Neural Imaging Techniques
Multi-modal imaging is a powerful approach for measuring brain hemodynamic properties. We used a single VCSEL light source with alternating light pattern output for simultaneous IOS and LSC imaging, enabling simultaneous comparisons as well as co-registration between imaging techniques. Monitoring the activity of neuronal populations is key to understanding the functional architecture of the brain. Optical imaging of hemodynamic correlates to brain activity, known as Intrinsic Optical Signal (IOS) imaging (for blood oxygenation levels) and Laser Speckle Contrast Imaging (LSCI) (for blood flow changes) are widely accepted techniques for obtaining maps of brain function in the cerebral cortex.

We have developed a high resolution multi-modal brain imaging system (florescence, IOS, LSCI) to investigate the relationship between blood flow, blood oxygenation/volume, intracellular calcium and electrographic activity in live rats during acute seizure-like events (SLEs), both before and after pharmacological intervention [1]. This imaging method is well-suited to studying pharmacological interventions and dynamic neurovascular changes during a disease.
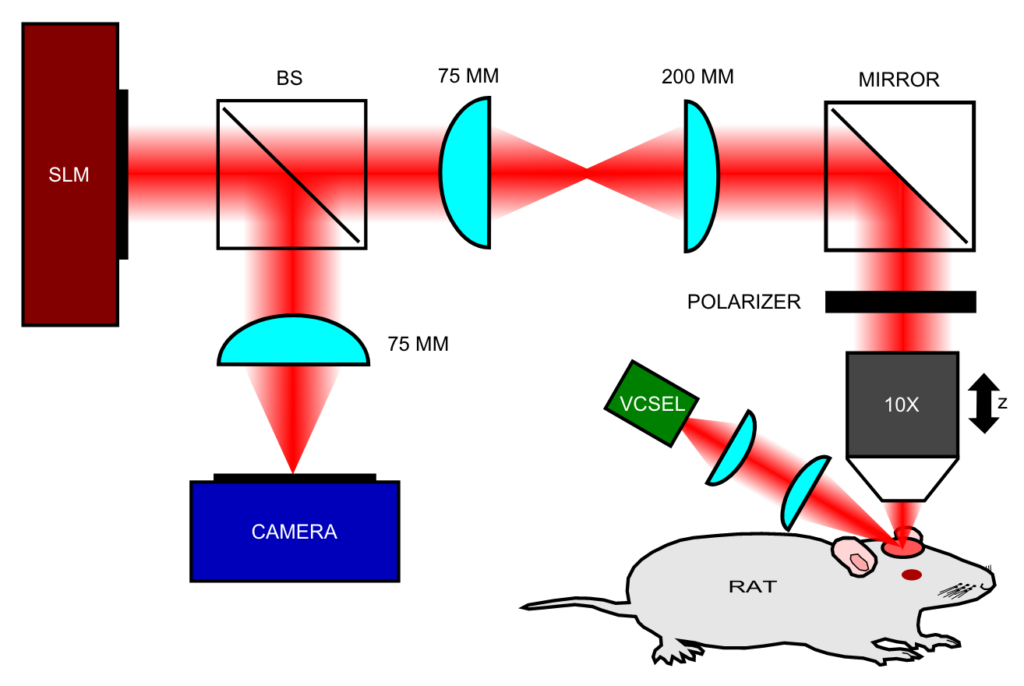
We have developed optical solutions to improve the robustness of such techniques, such as Laser Speckle Contrast Imaging (LSCI) technique with extended depth of field (DOF) [2]. We employed wavefront coding on the detected beam to gain quantitative information on flow speeds through a DOF extended two-fold compared to the traditional system. We characterized the system in-vitro using controlled microfluidic experiments, and applied it in-vivo to imaging the somatosensory cortex of a rat, showing improved ability to image flow in a larger number of vessels simultaneously.
We have also developed an autofocus algorithm; in order to obtain reliable quantitative data from LSCI the object must remain in the focal plane of the imaging system for the duration of the measurement session [3]. However, since LSCI suffers from inherent frame-to-frame noise, it often requires a moving average filter to produce quantitative results. This frame-to-frame noise also makes the implementation of rapid autofocus system challenging. We have demonstrated an autofocus method and system based on a novel measure of misfocus which serves as an accurate and noise-robust feedback mechanism. This measure of misfocus is shown to enable the localization of best focus with sub-depth-of-field sensitivity, yielding more accurate estimates of blood flow speeds and blood vessel diameters.
References
- D. Ringuette, M. A. Jeffrey, S. Dufour, P. L. Carlen, and O. Levi, “Continuous multi-modality brain imaging reveals modified neurovascular seizure response after intervention,” Biomed. Opt. Express 8, 873–889 (2017).
- I. Sigal, R. Gad, A. M. Caravaca-Aguirre, Y. Atchia, D. B. Conkey, R. Piestun, and O. Levi, “Laser speckle contrast imaging with extended depth of field for in-vivo tissue imaging,” Biomed. Opt. Express, BOE 5, 123–135 (2014).
- D. Ringuette, I. Sigal, R. Gad, and O. Levi, “Reducing misfocus-related motion artefacts in laser speckle contrast imaging,” Biomed. Opt. Express 6, 266 (2015).
Portable Brain Imaging
We have developed a miniature label-free imaging system for monitoring brain blood flow and blood oxygenation changes in awake, freely behaving rats. The device, weighing 15 grams, enables imaging in a ∼ 2 × 2 mm field of view with 4.4 μm lateral resolution and 1 − 8 Hz temporal sampling rate. The imaging was performed through a chronically-implanted cranial window that remains optically clear between 2 to > 6 weeks after the craniotomy. This imaging method is well suited for longitudinal studies of chronic models of brain diseases and disorders. In this work, it is applied to monitoring neurovascular coupling during drug-induced absence-like seizures 6 weeks following the craniotomy.
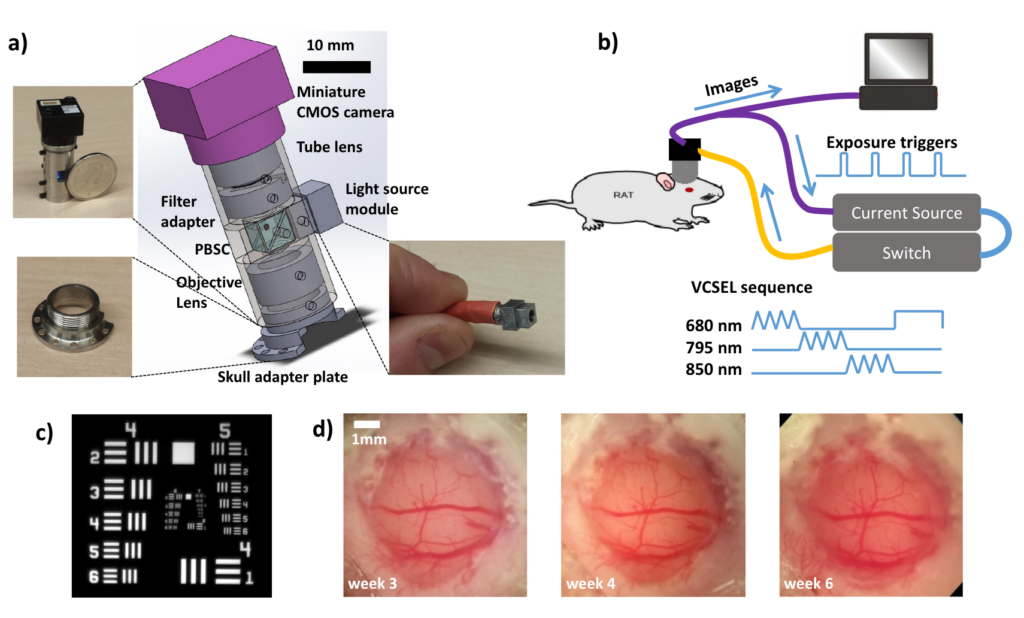
Miniature head-mounted imaging system for monitoring rat brain hemodynamics. a) Device schematic. Insets: the imaging tube (top left), skull adapter plate (bottom left), and the light source module (bottom right). b) System diagram. c) Image of a USAF resolution target: computed contrast values are 11.5% at 161 lp/mm, 15% at 144 lp/mm, 19% at 128 lp/mm. d) Brain imaged through the chronic cranial window. From [1].
References
- I. Sigal, M. M. Koletar, D. Ringuette, R. Gad, M. Jeffrey, P. L. Carlen, B. Stefanovic, and O. Levi, “Imaging brain activity during seizures in freely behaving rats using a miniature multi-modal imaging system,” Biomed. Opt. Express 7, 3596–3609 (2016).
Semiconductor Biosensor Design
Current Projects
Ultrasound Sensors
We are currently developing photoacoustic imaging (PAI) sensors based on highly-sensitive silicon photonics (SiP) sensor designs.

We have also developed photonic crystal slabs (PCSs) designs which offer a miniaturized platform for wideband, sensitive ultrasound detection by exploiting the photoelastic effect in water. However, poor modal overlap with the sensing medium and non-negligible absorption loss of the aqueous medium have previously limited PCS sensor performance. In this study, we detail the development and optimization of a PCS-based acoustic sensor by adding to it a low-loss high-index polymer cladding layer. Exploiting a mode-optimized TM-like optical resonance present in a PCS, with high bulk index sensitivity (RIU>600nm) and quality factor Q (>8000), we demonstrate real-time ultrasound sensing at a noise equivalent pressure of 170 Pa (1.9Pa/Hz−√1.9Pa/Hz). The PCS sensor is backside-coupled to an optical fiber, which, along with its intensity-based ultrasound-sensing architecture, will allow us to scale up easily to a 2D array. This work paves the way to a sensitive compact ultrasound detector for photoacoustic-based diagnostics and monitoring of tissue.
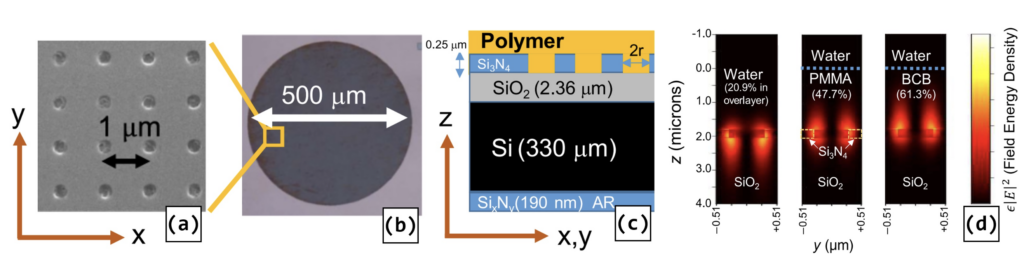
Hole-Array Polymer-Coated SiNx PCS Devices. a) unit cell lattice. b) device footprint c) layer cross-section. d) cross-section electric field energy density distribution.
(Text and figure from [1]).
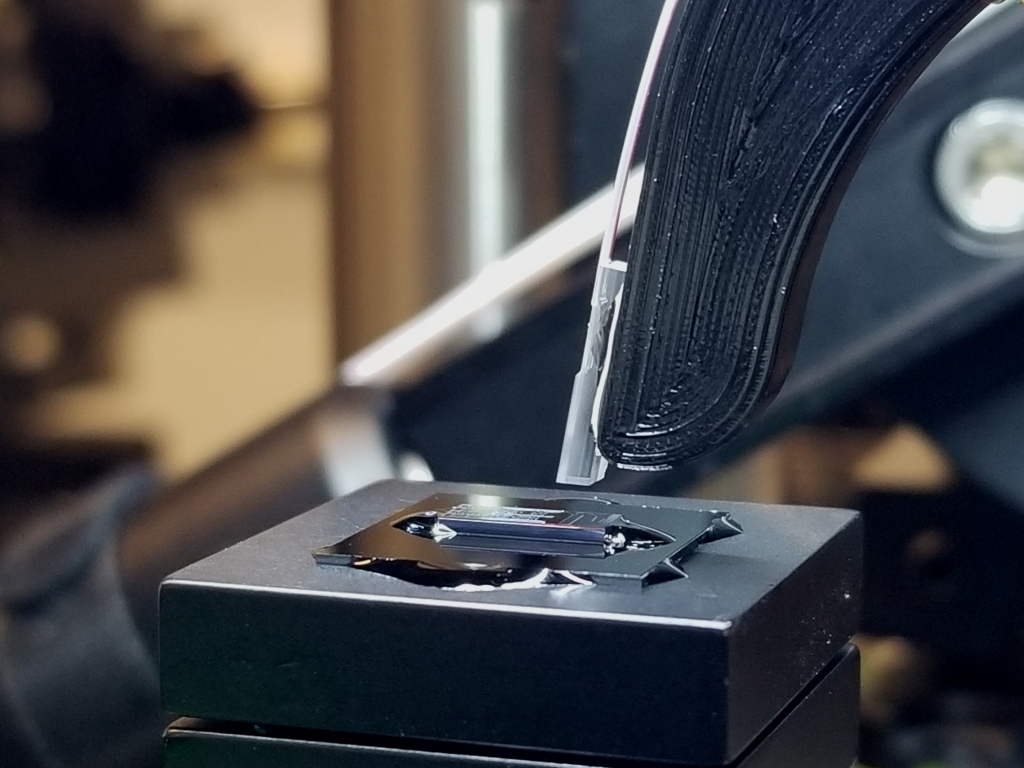
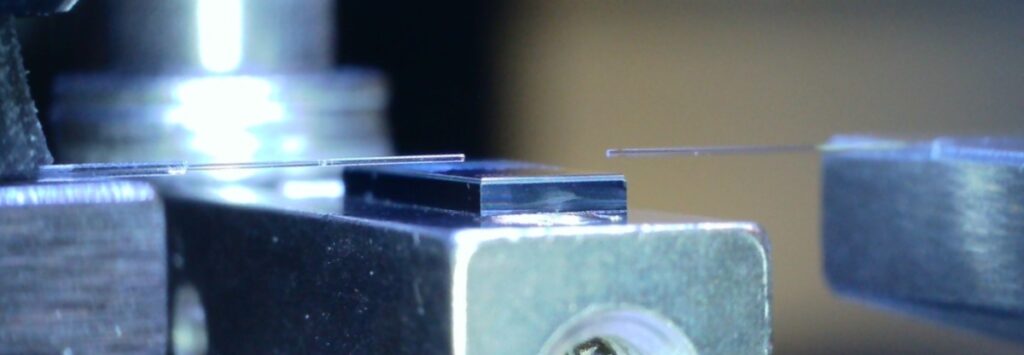
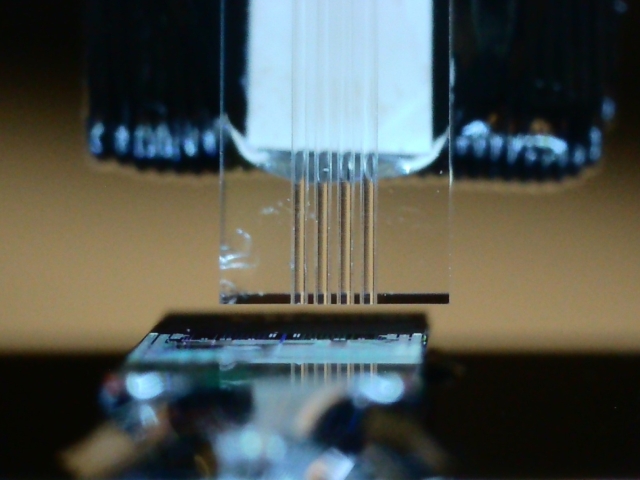
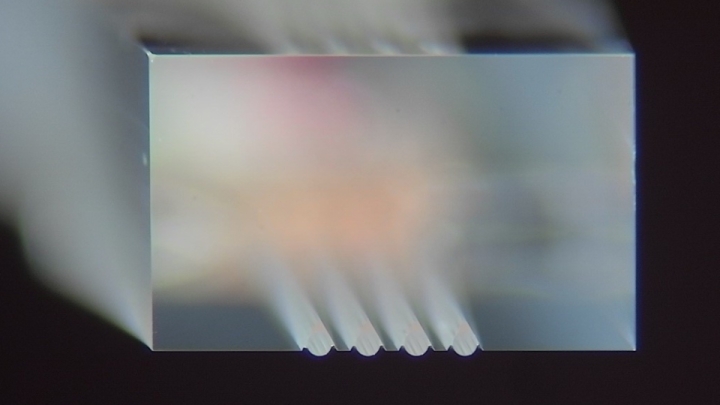
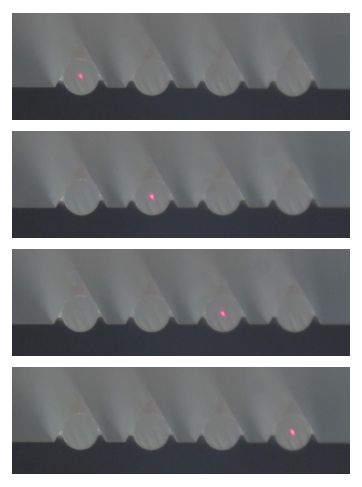
Silicon photonic chip interrogation setup and fiber arrays.
References
- E. Y. Zhu, M. Charles-Herrera, C. Rewcastle, R. Gad, L. Qian, and O. Levi, “Real-time ultrasound sensing with a mode-optimized photonic crystal slab,” Opt. Lett. 46, 3372–3375 (2021).
- E. Y. Zhu, C. Rewcastle, R. Gad, L. Qian and O. Levi, “Refractive-Index-based ultrasound sensing with photonic crystal slabs”, Optics Letters 44(10) pp. 2609-2612 (2019).
Past Projects
Semiconductor Biosensors
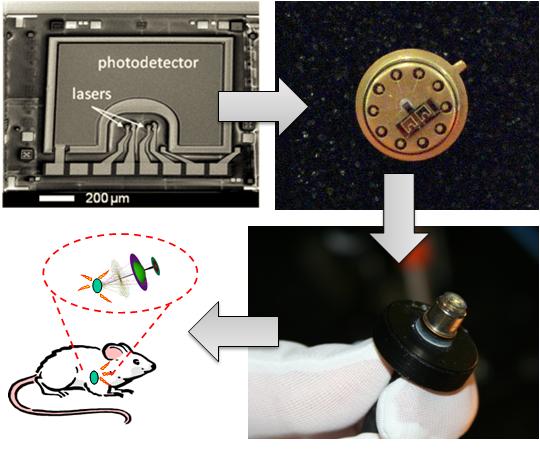
Optical techniques are widely used in clinical settings and biomedical research labs. These techniques include the study of fluorescence, bioluminescence, absorption, and scattering to determine changes in bio-molecular interactions. The majority of optical bio-sensing and bio-imaging techniques use commercially available lasers, detectors, lenses, spectrometers, and microscopes. Advances in microelectronics and optoelectronics fabrication techniques allow miniaturization of optical imaging and scanning systems and tailored light sources and detectors. Robust fabrication techniques in Silicon (Si) and Gallium Arsenide (GaAs) devices are increasingly used to create many semiconductor-based lasers, detectors, and waveguides optimized for biomedical applications. Integrated imaging systems can offer new possibilities for fundamental studies and future bio-medical and bio-defense applications. For example, the tremendous reduction in size, power, and cost of an integrated system enables long term investigations on freely-behaving, unanesthetized animals that are impossible with existing technologies.
We developed novel optical techniques based on a fully-integrated sensor as a building block for bio-sensing and bio-imaging. Key enabling technologies for these techniques are micro-fluidics, miniature optics, VCSELs, Si and GaAs-based detectors, and CMOS circuits that can be integrated together to perform optimized bio- sensing, bio-imaging, and bio-molecular dynamics studies. An example of the fully-integrated sensor module is shown. We can design the sensor to operate at a desired wavelength within the near-infrared (near-IR) to provide low tissue absorption and low bio-molecular autofluorescence. Consequently, we can achieve deep tissue penetration and low background emission levels for improved sensitivity.
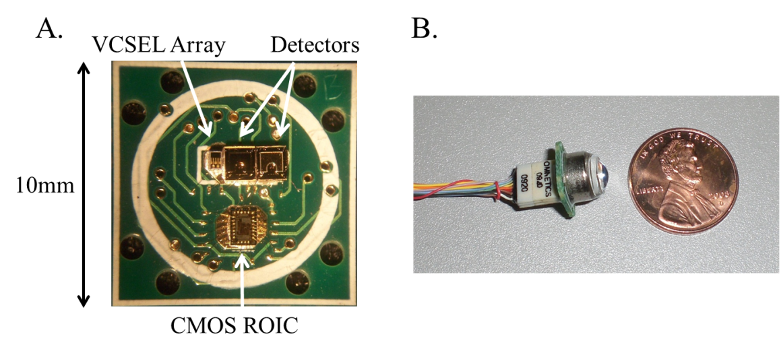
References
- T. D. O’Sullivan, E. A. Munro, N. Parashurama, A. De la Zerda, C. Conca, S. S. Gambhir, J. S. Harris, and O. Levi , “Implantable semiconductor biosensor for continuous in vivo sensing of far-red fluorescent molecules”, Optics Express 18(12), pp. 12513-12525 (2010)
- N. Parashurama, T. D. O’Sullivan, A. De La Zerda, P. El Kalassi, S. Cho, H. Liu, R. Teed, H. Levy, J. Rosenberg, Z. Cheng, O. Levi, J. S. Harris, and S. S. Gambhir “Continuous sensing of tumor-targeted molecular probes with a Vertical Cavity Surface Emitting Laser based biosensor”, J. Biomed. Opt. 17(11), pp. 117004 (2012)
- T. D. O’Sullivan, R. T. Heitz, N. Parashurama, D. B. Barkin, B. A. Wooley, S. S. Gambhir, J. S. Harris, and O. Levi, “Real-time, continuous, fluorescence sensing in a freely-moving subject with an implanted hybrid VCSEL/CMOS biosensor,” Biomed. Opt. Express 4(8), pp. 1332-1341 (2013).
Nanobiosensing
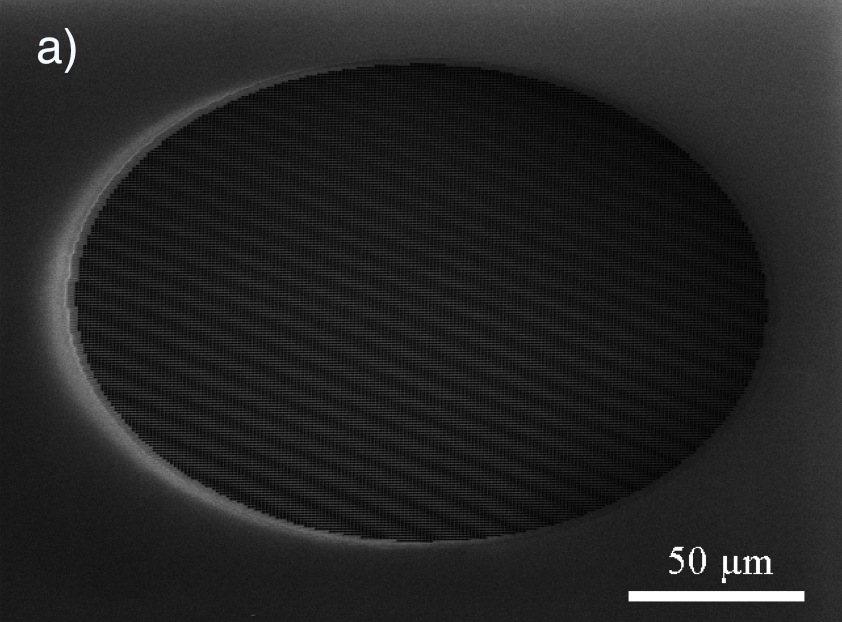
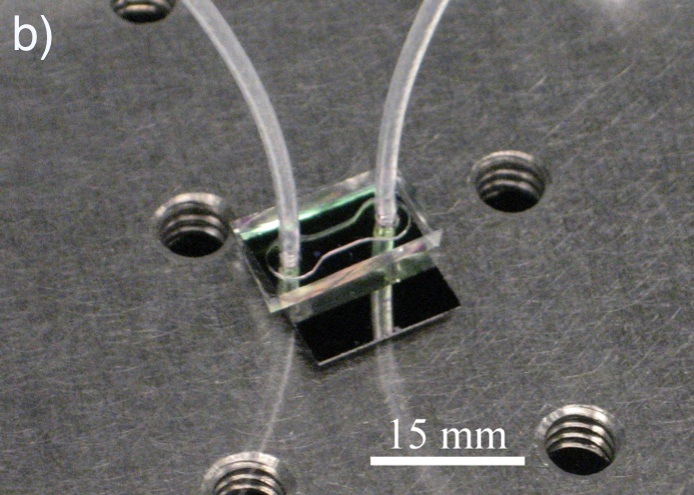
The use of photonic crystals as biosensors has gained considerable attention in recent years due to their compact size and attractive sensing characteristics. Photonic crystal slabs (PCS) incorporate these valuable properties with the ability to couple light from free-space radiation modes into guided resonances by vertical light coupling. PCS-based sensors operate by detecting minute changes in the index of refraction of a surrounding medium by observing shifts in guided resonance frequencies. In the context of biosensing, a PCS integrated in a microfluidic flow channel may be functionalized with biorecognition (capture) molecules to detect small changes in index of refraction incurred by bound analytes, eliminating the need for external labels or “tags”. Label-free approaches allow for rapid real-time detection, while minimizing sample preparation and potential interference with analytes.
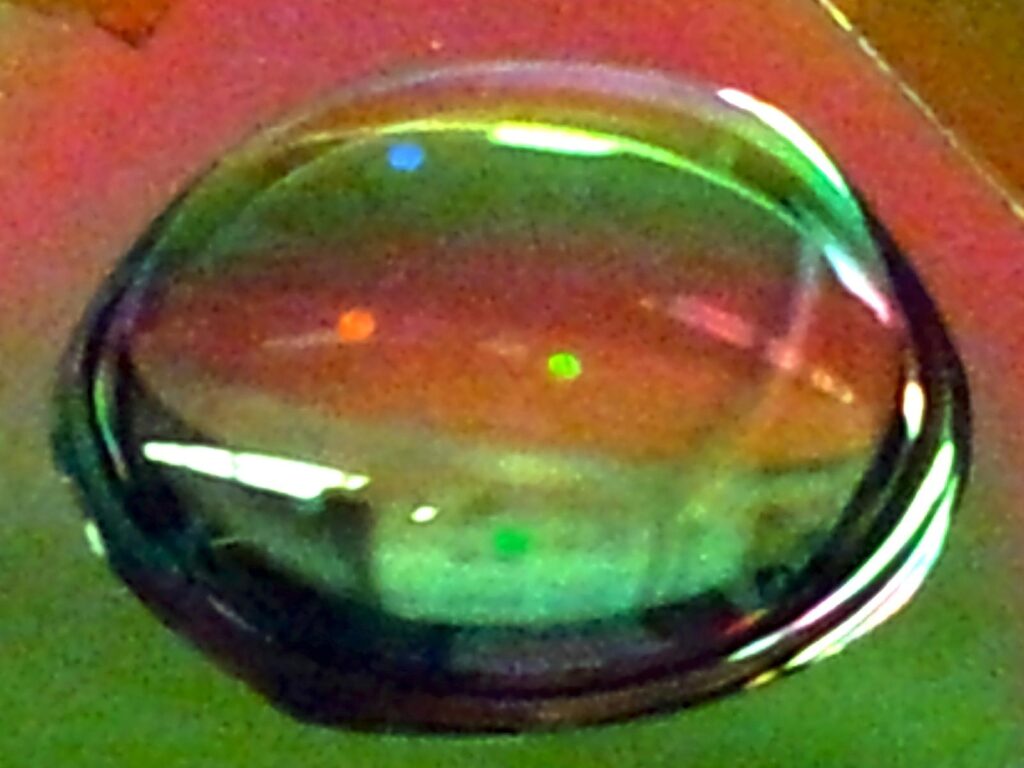
in the lattice parameters of each mesa.
References
- M. El Beheiry, V. Liu, S. Fan, and O. Levi, “Sensitivity Enhancement in Photonic Crystal Slab Biosensors”, Optics Express, 18(22), 22702-22714 (2010).
- C. Nicholaou, W. T. Lau, R. Gad, H. Akhavan, R. Schilling, and O. Levi, “Enhanced Detection Limit by Dark Mode Perturbation in 2D Photonic Crystal Slab Refractive Index Sensors”, Optics Express, 21(25), pp. 31698-31712 (2013).
- R. Gad, W. T. Lau, C. Nicholaou, S. Ahmadi, I. Sigal and O. Levi, “Tailoring of spectral response and spatial field distribution with corrugated photonic crystal slab”, Optics Letters, 40(16) pp. 3715-8 (2015).
Biosensor Applications
Current Projects
Photoacoustic Sensing
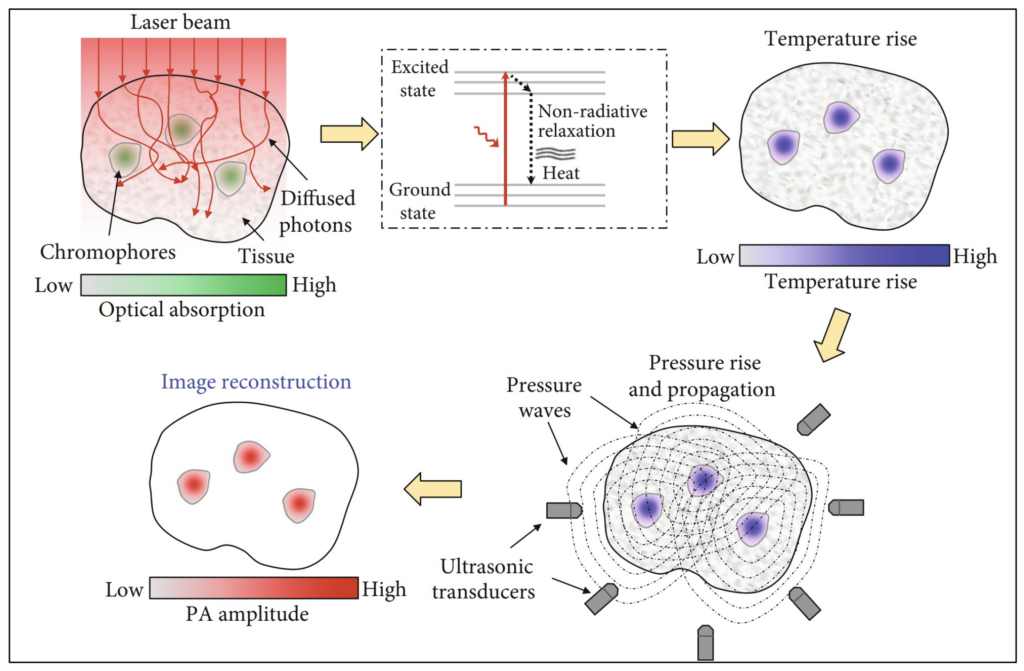
Photoacoustics (PA) is an imaging method that combines the benefits of both the ultrasound and optical imaging modalities. When tissue is stimulated by high intensity laser pulses (or EM radiation) localized tissue heating and cooling results in the formation of ultrasound waves (travelling pressure waves), allowing for deeper tissue imaging.
We aim to develop sensitive optical sensors for in vivo tissue imaging, with the ultimate goal of creating a clinical PA imaging system. Moreover, our sensitive sensor designs can allow for real-time therapy monitoring during surgeries or radiation therapy treatments. Our current sensor designs leverage silicon photonics fundamentals to integrate multiple optical devices (photonic integrated circuits) onto a single chip. We use specialized optical device simulation tools, clean room fabrication processes, and advanced characterization techniques to evaluate the optical and acoustic properties of fabricated devices. We collaborate with US hospital research teams and industry professionals to apply our achievements towards improving patient outcomes.
References
- L. Li and L. V. Wang, “Recent advances in Photoacoustic tomography,” BME Frontiers, vol. 2021, pp. 1–17, 2021.
Past Projects
Implantable in-vivo Monitoring
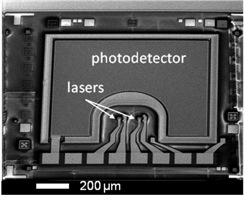
Medical imaging has evolved in recent years from a means to diagnose and evaluate large anatomical changes into a highly specialized tool used by physicians and scientists for the interrogation of events at the cellular and molecular level in living patients. Molecular imaging has enabled the medical community to diagnose and monitor diseases based on specific molecular events. Thus, molecular functional imaging represents a shift in the paradigm of experimental planning, whereby assays are no longer relegated to a test tube or cells in culture, but can be performed in a physiologically-relevant setting. Optical in vivo functional imaging has several advantages over other modalities. It is relatively inexpensive, versatile, sensitive, quick, and easy to implement. One of its drawbacks, however, is its limited ability to easily scale to human imaging due to the scattering and absorption properties of living tissue. This currently limits optical imaging techniques to shallow tissues (a few cm deep) or endoscopy-based probing. Implanting integrated sensors to report on fluorescence from functional imaging probes can overcome this limit.
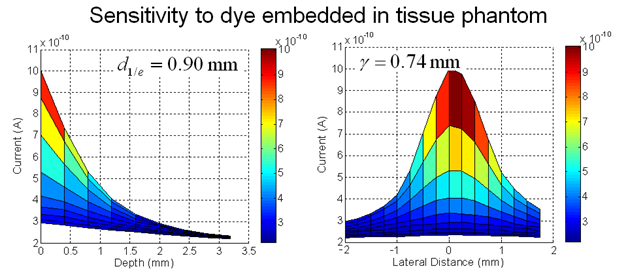
We are developing the instruments and techniques to accomplish portable, live animal monitoring using implantable sensors. Integrated VCSEL source/PIN photodiode detector chips can be used for fluorescent sensing with filters optimized for specific dye wavelengths. We are currently using devices optimized for use with Cy 5.5 (670 nm/720 nm), but flexible enough to be applied in a number of different dye schemes. Characterization of these devices has shown that signal can be obtained from dye present several millimeters within tissue.

vein with 3 nmol of cRGD-Cy5.5 (N ¼ 3, left) or 3 nmol cRAD-Cy5.5 (N ¼ 3, right) acquired using a cooled CCD camera. From [1].
Molecular beacons can be used as functional biomarkers to target specific proteins, potentially revealing the presence of cancerous tissues. We are working with Pyroeophorbide acid-based markers to target fibroblast activation protein (FAP), present only in epithelial tumour stroma. By studying the time course uptake of the marker within a tumour we can learn about metabolization in cancerous tissues, as well as investigate the efficacy of potential treatments such as photodynamic therapy (PDT). Implantable biosensors allow for the continuous monitoring of awake animals, removing the limits inherent to imaging under anaesthesia, and introducing the possibility of long term monitoring over days or weeks.
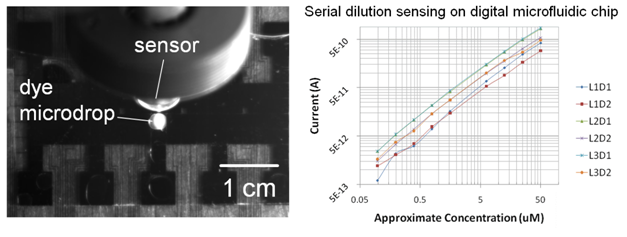
We are currently investigating other uses of these biosensors, including integration with digital microfluidic devices. The inclusion of a reliable portable sensor with such instruments can allow for real time quantification of reaction dynamics, and lead to better monitoring and control of small volume reactions.
References
- Natesh Parashurama, Thomas D. O’Sullivan, Adam De La Zerda, Pascale El Kalassi, Seongjae Cho, Hongguang Liu, Robert Teed, Hart Levy, Jarrett Rosenberg, Zhen Cheng, Ofer Levi, James S. Harris and Sanjiv S. Gambhir. “Continuous sensing of tumor-targeted molecular probes with a vertical cavity surface emitting laser-based biosensor.” Journal of Biomedical Optics, 17(11): 117004-117004 (2012).